Introduction
The human immunodeficiency virus (HIV) weakens the immune system of the patient, which leads to a declined immune response, and makes the patient prone to many kinds of infections, diseases, and even cancer, resulting in acquired immunodeficiency syndrome (AIDS) (1). Researchers believed that HIV was transmitted to humans by chimpanzee’s immunodeficiency virus from west Africa called simian immunodeficiency virus (SIV) and mutated as HIV during the period of hunting chimpanzees for meat (2). It started to spread at a slow rate across Africa, which was then established globally. HIV is transmitted from one person to another through infected blood, vaginal fluids, semen, shared drug needles, mother to baby during pregnancy, breastfeeding, etc. (3–5). HIV is a retrovirus categorized under the class of RNA viruses that inserts its genetic material into human cells for replication (6). The size of the HIV is ∼145 nm in diameter with a spherical shape (7). The structure of HIV is shown in Figure 1. The HIV genome has 2 kinds of genes, such as structural genes and regulatory genes. Structural genes are group-specific antigen (gag), DNA polymerase (pol), and envelope (env). Regulatory genes are trans-activator of transcription (tat), regulator of virion expression (rev), negative regulatory factor (nef), viral infectivity factor (vif), lentivirus protein R (vpr), and viral protein U (vpu) (8–13). Also, HIV has many proteins and enzymes which help them with virus replication in the host, which are summarized in Table 1.

Figure 1. Structure of HIV (14).
There are 2 types of HIV, namely HIV-1 and HIV-2, where HIV-1 is commonly referred to as HIV and is highly virulent. In contrast, HIV-2 is a less virulent strain that is found only in west Africa depletes the immune system at a very
low rate, and originated from SIV of the sooty mangabey monkey (26). HIV-1 has different kinds of strains, such as major (M), new (N), and outlier (O) groups. Group M is a pandemic virus strain that emerged from Kinshasa in the 1920s (27). HIV can be diagnosed with the common earlier symptoms such as fever, headache, sore throat, joint pain or muscle aches, skin rash, swollen lymph nodes, etc., which lasts up to 1–2 weeks (28). After many years of infection, symptoms such as swollen lymph nodes, weight loss, extreme tiredness, fever, night sweats, etc. start to show up and persist (29). Further, diagnosing can be done in hospitals to confirm the presence of a virus through antibody or antigen tests conducted for the samples such as urine, saliva, blood, etc. There are 3 stages of HIV infection, such as stage 1—Acute HIV infection, stage 2—clinical latency (HIV inactivity or dormancy), and stage 3—AIDS (30). During stage 1, an HIV-infected patient develops a persistent flu-like illness within 4 weeks postinfection. Stage 2 is known as asymptomatic or chronic HIV infection. In this stage, HIV is found active but replicates slowly. At stage 3, patients with AIDS experience severe illnesses called opportunistic illnesses due to the weakening of the immune system (31). Understanding the replication process can improve the treatment technologies and eventually find a cure for the disease.
HIV replication
Step 1 – Viral Attachment: HIV binds to the immune cell by the attachment of glycoprotein Gp-120 to the CD4 protein receptor and either the CCR5 or CXCR4 co-receptor on the immune cell (32).
Step 2 – Entry: Fusion of viral envelope on cell membrane makes the way for easy entry of HIV capsid into immune cells.
Step 3 – Replication: After the entry of the HIV capsid into the cell, the reverse transcriptase enzyme, which has ribonuclease activity, degrades RNA while synthesizing cDNA. Further, the reverse transcriptase enzyme makes copies of cDNA. DNA polymerase makes coding DNA from noncoding and coding complexs present in cDNA. cDNA and the complement of cDNA forms into double-stranded viral DNA and moves to the immune cell nucleus. Viral DNA is incorporated into the genome of immune cells by the assistance of the integrase enzyme. Integrated viral DNA remains dormant in the latent stage of HIV infection.
Step 4 – Biosynthesis: The HIV-1 mRNA was translated through a multifaceted process of translation initiation that was facilitated by both cap-dependent and internal ribosome entry site (IRES)-driven mechanisms during the biosynthesis of HIV replication. Cellular factors, such as heterogeneous nuclear ribonucleoprotein, have a significant impact on enhancing the function of the HIV-1 5 untranslated region IRES. These factors shuttle from the nucleus to the cytoplasm, where they assist in the initiation of IRES-mediated translation. Factors such as Rev are crucial in mRNA export, polysomal association, and overcoming translational inhibition. Furthermore, influencing HIV-1 IRES activity in the cytoplasm involves variations associated with specific IRES transacting factors. Early in the infection, the virus shows its ability to alter host machinery to generate viral proteins and finally release infectious virions by competing with cellular machinery for ribosomes and eukaryotic translation initiation factor (33).
Step 5 – Assembly: Coordinating the production of viral particles depends significantly on the gp120 glycoprotein in the HIV envelope reaching the cell membrane. The method comprises the attachment of viral RNA, Gag (p55), and Gag-Pol (p160) polyproteins, to the plasma membrane. This interaction triggers the initiation of the development of an immature viral bud. This undeveloped structure lacks the ability to spread and causes the bending of the membrane, mostly because of the existence of unprocessed Gag polyproteins. The viral protease enzymes play a crucial role in the cleavage of Gag, which is necessary for the development of the virion. The occurrence of this cleavage event initiates a sequence of structural changes that result in the formation of fully developed HIV particles. The mature virions are composed of specific structural elements, such as the matrix, capsid, and nucleocapsid proteins, which play a crucial role in the virus’s ability to infect and replicate (34).
Step 6 – Release: Mature HIV virus released from the cell starts infecting other cells.
Likewise, HIV starts infecting gradually to other cells and enters the brain, causing neuro AIDS. The complete replication of HIV is illustrated in Figure 2.

Figure 2. Pathway of HIV replication (35).
HIV in brain
HIV-1 infection spreads to the central nervous system (CNS) and peripheral nervous system (PNS) causing 30–50% of patients to have neuro AIDS which leads to a decline in brain functions like movement, change in behavior, and mood (36). This condition is termed HIV associated neurocognitive disorder (HAND). HAND is classified into asymptomatic HAND/asymptomatic neurocognitive impairment, sHIV associated dementia, and minor neurocognitive disorder (37). The virus present in the brain compartment cannot be completely eradicated because of the HIV-associated neurocognitive diseases was caused in part by the persistence of HIV within the CNS, neurotoxicity, difficulties in therapy penetration, individual variability in disease development, and lack of consistent treatment protocols (38). So, understanding the mechanism behind the migration of HIV infection across the blood brain barrier (BBB) is very much required.
Migration of HIV across BBB
The entry of monocytes/macrophages into the brain occurs as a result of the disturbance of endothelial tight junction proteins, namely occludin and zonula occludens-1. The HIV Tat protein stimulates astrocytes and microglia to produce an excessive number of chemokines, which in turn leads to the infiltration of leukocytes into the CNS (Figure 3). Similarly, Gp120 plays a crucial role in the development of nerve damage and programmed cell death in HIV infection (40). A remarkable function of the Tat protein as a stimulant for growth has been suggested, leading to the movement of HIV-infected cells across cell barriers and into the CNS. NeuroAIDS was not influenced by the level of HIV in the body and instead is associated with the extent of monocyte infiltration, which occurs after the activation of microglia in the brain. HIV-1 targets exclusively CD4-expressing cells with either CXCR4 or CXCR5. HIV-1 strains that specifically target CXCR5 mostly infect macrophages and are therefore categorized as M-tropic. Conversely, strains that target CXCR4 primarily infect T cells and are classified as T-tropic (41). HIV-1 R5 strains that are macrophage-tropic (mac-tropic) have the ability to infect macrophages that have low levels of CD4 expression. Conversely, nonmacrophage tropic (nonmac-tropic) HIV-1 R5 variants mostly target CD4+ T cells and need a high degree of CD4 expression. The brain tissue is mostly occupied by HIV-1 R5 variations that have a strong affinity for macrophages. These variants can be found in the CSF, but their presence in immunological tissue or the blood is rare, especially in advanced stages of the disease. The primary HIV reservoir in microglia and macrophages is established by adapting HIV-1 R5 envelopes (mac-tropic HIV-1 R5 envs) to replicate in these cells. This is due to the presence of these envelopes in brain tissue. Furthermore, the process by which the HIV-1 virus spreads from infected macrophages and forms a reservoir can be elucidated as the result of direct interactions between infected T cells and macrophages. This interaction leads to the merging of cells, facilitating the rapid and extensive transmission of R5 tropic viruses to macrophages. The merged cells possess the capability to amalgamate with adjacent noninfected macrophages, resulting in the formation of multinucleated giant cells (42).

Figure 3. Migration of HIV-1 across blood brain barrier (39).
HIV conventional treatments
Anti-retroviral therapy (ART)
During the year 1964, there was a claim that azidothymidine (AZT) or zidovudine, belonging to the class of nucleoside reverse transcriptase inhibitors (NRTIs), was a potential drug for cancer therapy, but it did not get approval as it is not effective against cancer (43). However, in the 1980s, the National Cancer Institute conducted a screening test of AZT against HIV/AIDS and found it effective. The British pharmaceutical company Burroughs Wellcome evaluated the AZT drug against AIDS and found that it declined death and opportunistic illness (44). In March 1987, AZT received approval from the U.S. Food and Drug Administration as the first drug to combat AIDS (45). Through further research on AZT, the AIDS clinical trial group (ACTG) 016 clinical trial found that by lowering the therapeutic dosage, serious side effects of the drug can be reduced. The ACTG 019 clinical trial evaluated the benefits of AZT that if administrated before stage 3, there is a considerable delay in progression to AIDS (46). Further, to improve the efficacy of the treatment, ART is provided with a combination of drugs.
Two-drug therapy or combination ART (cART)
During ART treatment, the replication of HIV results in mutation. These mutations will alter the virus properties and display resistance to ART drugs. Hence, researchers started to evaluate the effect of combining drugs and develop an environment that becomes difficult for the virus to resist. During that time, a study funded by the National Institute of Allergy and Infectious Disease (NIAID) examined the impact of combining AZT with another NRTI drug called Dideoxycytidine (ddC). The results demonstrated that this combination was more effective than using AZT alone (47).
Three-drug therapy or highly active antiretroviral therapy (HAART)
In 1996, scientists made a significant discovery regarding the effectiveness of triple-drug therapy in suppressing the replication of HIV, specifically targeting a mutant strain of the virus. A successful version of ART by triple drug therapy is highly efficient and is termed HAART (48). The high efficiency may be due to the discovery of a new class of protease inhibitor ART drug: saquinavir. The protease inhibitor got its first approval from the FDA in 1995. In the year 1996, NIAID supported a clinical trial to evaluate the efficacy of HAART over cART and showed the combination of saquinavir, ddC, and AZT showed better performance than ddC and AZT. This three-drug combination has the ability to reduce the viral count in blood to an undetectable range for 1 year for patients who have a history of taking AZT alone. However, there will be also a few active forms of virus proliferation that remain intact during HAART. This is caused due to the skipping of drugs by the patients during treatment since HAART is a lifelong treatment (49). Currently, HAART serves as an inhibitor for the replication process, reverse transcription, protein maturation, and the integration of viral DNA into host chromosomes (50). This action activates the viral proliferation, i.e., replication other than intentions that cannot be followed by the physicians. In a clinical trial, researchers found that missed doses cause neurocognitive defects and issues such as amnesia, insomnia, infirmity, and depressed.
Further development in therapy
The limitations of drugs, such as toxicity and drug resistance development, are still present. Researchers have been aware that CD4 is the primary HIV receptor on immune cells since the 1980s. However, in the mid-1990s, researchers discovered that HIV strains used CXCR4 as a co-receptor to enter immune cells. Researchers, driven by inspiration, conducted a thorough search for additional co-receptors and discovered that HIV also uses CCR5 as a co-receptor to infect immune cells (51). The FDA approved the advancement of maraviroc, a medication that blocks the CCR5 receptor, in 2007. In 2007, researchers discovered a significant class of drugs known as integrase inhibitors (52), such as raltegravir, which they used in conjunction with ART. However, HIV can develop resistance to these drugs through various pathways. Therefore, there might be a chance of developing resistance to the first-generation integrase inhibitor (elvitegravir). In 2013, the second generation of integrase inhibitors got approval from the FDA, and the drug name is dolutegravir, which shows the greatest barrier to the development of drug resistance in HIV. The clinical trial evaluation found that dolutegravir was effective against patients who had not taken HIV therapy but ineffective against those who had treatment, including those who took first-generation integrase inhibitors. Dolutegravir has additional advantages, such as convenience for daily dosing, safety, and low production costs. Table 2 summarizes the many ART drugs currently available.
Disadvantage of using conventional treatments
Daily dosage for patients causes inconvenience since it has no complete cure and has to intake throughout their lives, causing side effects (53). Even though conventional treatment is administered latently (the resting state where it remains silent and does not produce new HIV but anytime it can be active and produce more HIV), HIV escapes from the treatment and exists in the cell reservoir. Cells that hide as inactive forms remain in secondary lymphoid tissues, the CNS, lymph nodes, or the lungs and cannot be accessed by antiretroviral drugs. The anti-retroviral drugs still have drawbacks like low solubility and poor bioavailability (54). The continued intake of drugs for a prolonged time leads to the development of drug resistance by HIV.
Challenge in treatment of neuro-AIDS
The primary challenges are the complex structure of BBB, weak biodistribution, and poor pharmacokinetics. The presence of a large number of mitochondria and a low amount of pinocytotic activity in brain microvessel endothelial cells declines the delivery of antiretroviral drugs across the BBB (55). Moreover, the upregulation of p-glycoprotein (p-gp) impedes the penetration of several types of medications, including protease inhibitors, antiepileptic pharmaceuticals, and anti-inflammatory compounds, across the BBB (56). For a novel therapeutic approach, a drug delivery system should possess properties like site targeting, enhanced drug penetration, and effective drug delivery. To attain these properties, the concept of targeted drug delivery comes into existence, where nanocarrier helps the drug to reach the HIV-infected cells and deliver it.
Role of nanotechnology in HIV treatment
To achieve effective treatment for HIV-infected individuals, all HIV reservoirs must be removed. So, researchers have been developing drug delivery systems to reduce dosage, increase CNS efflux, and enhance site-specific delivery to improve efficiency with limited toxic effects of ART drugs (57). Here comes the advancement of nanotechnology towards the development of novel nanotechnology-based ART drug delivery techniques that display declined side effects. There are various kinds of nanotechnology-based approaches that can enhance the delivery of ART drugs (nano-ART) across the BBB, leading to the increased biodistribution and efficacy, which includes solid lipid nanoparticles, polymeric nanoparticles, nanogels, nano-emulsions, nano-suspensions, nano-spheres, nano-micelles, liposomes, lipid drug conjugates, nanostructured lipid carriers, etc. (58, 59). These nanotechnology carriers may administer existing HIV medications in a regulated and/or targeted way, therefore enhancing drug bioavailability and prolonging residence duration at target areas, significantly improving the quality of life for HIV patients (60). Owing to the better results displayed by the targeted drug delivery, various nano carriers are under preclinical trials for the treatment of neuro aids (54, 61). Some of the nanocarriers utilized for ART drug delivery are discussed below.
Liposome based drug delivery system
Liposome is one of the majorly used nano-drug targeting/ delivery systems owing to its low toxicity and long circulation stability (62). Liposomes can penetrate deep into the bi-lipid layer of the cell membrane through endocytosis, and drugs can be delivered at the targeted site, i.e., within the cells (63). Also, it was reported that the liposomes not only target the drug to a specific site but also protect the drug from the physiological environment (64). It is well known that the replication of HIV cells takes place in mononuclear phagocyte system (MPS), and liposomes are readily taken up by the macrophages because of its size range. The uptake of liposomes by the macrophages suggests that liposomes can act as the carrier for the anti-HIV agent (65). Oussoren et al., (66) prepared liposomes as a carriers for encapsulating the dideoxycytidine-5’-triphosphate (ddCTP) to target MPS. The efficiency of ddCTP-encapsulated liposomes was evaluated in-vivo conditions where the reduction in provirus DNA was noted. At first, the C57BL/6 mice were injected with LP-BM5 murine leukaemia virus which causes murine immunodeficiency syndrome (MAIDS), that is similar to HIV. For the treatment of MAIDS, free ddCTP drug was injected via intraperitoneal administration to LP-BM5-infected mice (control). Also, MPS-targeted liposome-encapsulated ddCTP was injected via intraperitoneal administration to LP-BM5-infected mice (specimen). The results showed that the control drug was quickly absorbed into blood circulation after administration and degraded by the body fluids. But, while injecting liposome-encapsulated ddCTP drug, considerable reduction of provirus DNA in spleen and bone marrow was observed. However, there was no reduction observed in lymph nodes, and also, other HIV-targeted cells such as CD4+ T lymphocytes, were not efficiently targeted by liposome carriers. These limitations can be overcome with the help of surface modification with molecules that can specifically target CD4+ T lymphocytes. Bronshtein et al., (67) conducted a study to explore a novel treatment strategy for treating HIV. The technique included directing cell-derived liposomes specifically towards cells infected with HIV. The liposomes were synthesized using cytoplasmic membranes derived from cells expressing CCR5, a human receptor for gp120, on the surface of virions and HIV-infected cells. Cell-derived liposomes demonstrated the ability to selectively target gp120-expressing HIV model cells, as seen in in vitro investigations. The efficacy of the system was demonstrated by encapsulating EDTA in liposomes where EDTA acts as an enzyme inhibitor to HIV-infected cells, exhibiting a cytotoxic effect specifically to HIV-infected cells. Liposomes produced from cells and containing EDTA resulted in a 60% decrease in the survival of cells expressing gp120 while having no impact on control cells that do not express gp120. In essence, this research presents evidence of the precise targeting and destructive impact of CCR5-conjugated cell-derived liposomes on gp120-expressing HIV model cells. This suggests a promising new therapeutic strategy for treating HIV.
Kenchappa et al. (68) used liposomes as carriers for loading efavirenz (EFV) and glutathione (GSH). The primary objective of the study was to elucidate the human monocytic leukemia (THP-1) cell viability and ROS scavenging behavior after intracellular intake of liposomes containing EFV (with and without GSH). The results substantiated that the use of liposomes increased the presence of EFV in the macrophages, improved the quenching of ROS by GSH, and considerably improved the cell viability when EFV and GSH were loaded (Figure 4). Kheoane et al., (69) targeted liposomes as a prospective means of increasing the bioavailability of anti-HIV drugs, including EFV. Existing combinations of anti-HIV medications are poorly absorbed due to several factors, including low solubility, fast elimination, and extensive first-pass metabolism. In this study, the incorporation of drug absorption enhancers (DAEs) into the liposomes was studied to raise the bioavailability of EFV. Liposomes composed of various lipid combinations, such as DOPC:CHOL and DSPC:CHOL:DPPC, demonstrated optimal outcomes regarding drug entrapment, release, and permeability. Despite this, certain formulations demonstrated reduced biocompatibility with hepatic cells. Nonetheless, the liposomes exhibited potential for sustained release and enhanced drug loading, positioning them as a promising platform for anti-HIV drug delivery.
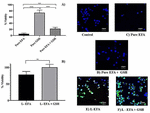
Figure 4. (A) Influence of pure EFA and GSH on cell viability, (B) Influence of liposome on the cell viability, (C–F) represent merged fluorescent images (68).
Polymer micelle-based drug delivery system for HIV treatment
Polymeric micelles are made up of block polymers that self-assembles as core-shell structures (70). They are homogenous systems, where polymer chains are arranged in a similar fashion as that of surfactants during micelle formation. A hydrophobic core and a hydrophilic shell form the architecture of polymeric micelles (71). Therefore, both hydrophobic and hydrophilic drugs can be efficiently loaded in polymeric micelles. The targeting ligands can be encapsulated within the carrier, or absorbed over the surface, or even linked to the surface of the carrier depending upon the nature of the ligand. Polymeric micelles can target HIV reservoir sites in macrophages through the opsonization process. Therefore, when the drug was encapsulated within the polymeric micelles, the drug will be released during the opsonization process, where infected macrophages will be delivered with encapsulated drug (72). Also, it was reported that the polymeric micelles have the ability to cross BBB and deliver anti-HIV medications through phagocytosis delivery (73).
Chiappetta et al. (74) studied the influence of micellar size, composition, and drug payload on the effects of different intranasal drugs targeting HIV in CNS. They examined simple micelles made of poloxamer F127 (a copolymer with high hydrophilicity) and mixed micelles made of 75% T904 and 25% F127 loaded with EFA. From the results, it was substantiated that the composition of the nanocarrier plays a crucial role in drug absorption from the nasal mucosa. Here, the T904 enhanced the encapsulation capacity of micelles, enabling more payload of the drug. This resulted in an increased bioavailability of EFA in the CNS. Another study by Chiappetta et al. (75) investigated the co-micellization of Pluronic F127, a poloxamer renowned for its strong hydrophilic properties, with the comparatively more hydrophobic poloxamines Tetronic T304 and T904. By combining these polymers in various weight ratios, a substantial improvement in solubility was achieved, with a remarkable increase from 4 μg/ml to over 33 mg/ml. Micelles containing drugs demonstrated enhanced physical stability over time in comparison to micelles composed solely of poloxamine. In the case of encapsulation efficiency (EE), mixed micelles were shown to be superior to pure counterparts F127 and T904. However, the F127 micelles with T304 resulted in a lower EE. The results of the investigation showed that the hydrophobicity of the system was much influenced by the presence of T304 and T904. The size and fluidity of the binary system were greatly influenced by the F127. Pediatric HIV treatment based on the poloxamer/poloxamine systems have demonstrated remarkable efficacy as nanocarriers for oral medication delivery.
Polymeric micelles can also be used as vaccines instead of drug delivery vehicles. Lamrayah et al. (76) employed polylactide-based block copolymers of 105 nm as a vaccine for HIV infection. Here, the antigenic protein p24 from HIV 1 was conjugated with the corona of polymeric micelles. Further, immunization activity of p24-conjugated micelles was studied against a standard mice model (Figure 5) and compared with gold standards and unconjugated micelles. The results substantiated that the p24-conjugated micelles displayed quantitatively improved immune response, indicating the potential of polymer micelles in vaccine development.

Figure 5. Fluorescence tomography used to track the distribution of fluorescent micelles over time after they were administered by the SC route in mice model (76).
Dendrimer based drug delivery system for HIV treatment
Dendrimers are branched macromolecules which can be loaded with drugs, targeting ligands, and imaging agents for effective diagnosis and treatment (77). For effective attachments of the drug molecules, dendrimers surface charge can be altered as positive, negative, and neutral. Dendrimers have three components: a central core, an interior shell to the core’s surrounding, and the surface (78). The 3D morphology of dendrimers mostly relies on the core, while the inner shells govern the host-guest characteristics. The surface functional groups possess an ionic charge and exhibit several reactive sites. The core structure and the number of branching units in the inner shell determined the shape of the dendrimers. The number of branching points determines dendrimer generations, with each layer of branching corresponding to a specific generation. The first layer of branching was referred to as the first generation (G1), the second layer as the second generation (G2), and so on. Generations are designated by the labels G1, G2, G3, etc. (79). Dinesh kumar et al., (80) synthesized PEGylated G4 and G5 polyamidoamine (PAMAM) dendrimers as carriers for the anti-HIV medication lamivudine. The G4 and G5 PAMAM dendrimers were synthesized by Michal addition and amidate reaction with an ethylene diamine core, and the surface was PEGylated using MPEG 4-nitrophenyl carbonate. Synthesized G4 or G5 PAMAM dendrimers were PEGylated by reacting them with M-PEG 4-nitrophenyl carbonate, and the resulting reaction mixture was dialyzed against distilled water for 72 h to give PEG-PAMAM dendrimers (Figure 6). The Lamivudine drug was incorporated into both PEGylated and non-PEGylated PAMAM dendrimers by dissolving them in methanol and then combining them with an aqueous solution of the medication. The elimination of unbound medication from the formulation was performed by dialyzing it in a cellulose dialysis bag using double-distilled water. When examining the physical and chemical characteristics of PEGylated and non-PEGylated PAMAM dendrimers, including UV, IR, TEM, DSC, drug entrapment, drug release, and hemolytic toxicity, it was found that PEGylation of PAMAM dendrimers decreases surface toxicity from 25% to 2%, enhances drug loading capacity, and extends the duration of drug release. As a result, PEGylated PAMAM dendrimers are well-suited for use as drug delivery vehicles.
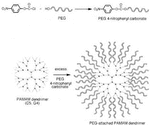
Figure 6. Schematic for the synthesis of PEGylated PAMAM dendrimers (80).
Daniel et al., (78) investigate the possibility of microbicides made from polyanionic carbosilane dendrimer-conjugated antiviral medicines stopping HIV transmission. Its main emphasis is on the proficiency with which Tenofovir (TFV) and Maraviroc (MRV) prevent infection. It highlights the structural advantages of dendrimers in enhancing medication transport and targeting many phases of the HIV life cycle, thereby increasing effectiveness and lowering resistance. The study employed in vitro assessments to evaluate the effectiveness of different dendrimer-drug combinations against R5 and X4 HIV-1 strains. A fixed ratio of 10:5:1 was used for G2-STE16/TFV/MRV, G1-S16/TFV/MRV, and G2-S24P/TFV/MRV. A method known as the Chou and Talalay method was used to assess drug interactions and calculate combination indexes (CIs) in order to determine if there was synergy. Additionally, clinical trials (MTN-007 and MTN-017) were referenced to investigate the safety and acceptability of TFV formulations. The results indicate that these unique combinations have the potential to greatly improve antiviral effectiveness, offering a hopeful approach to HIV prevention. Royo-Rubio et al., (81) examined the use of PEGylated cationic dendrimers for the delivery of microRNAs (miRNAs) as a prospective treatment strategy against HIV-1 infection. The study emphasizes the drawbacks presented by HIV-1, including treatment resistance and the capacity of the virus to form reservoirs in different cell types. The findings indicate that certain anti-HIV-1 miRNAs, when combined with dendrimers (called dendriplexes), are highly effective in stopping HIV-1 infection. This opens up the possibility of creating new treatments to fight the virus. The study shows that dendrimers can successfully capture and shield miRNAs, making them more stable and better at reaching the cells they need to target. This is a new and innovative approach. The research highlights the importance of developing advanced drug delivery methods to overcome the challenges in managing HIV-1.
Niosomes-based drug delivery system for HIV treatment
Niosomes are similar to liposomes, as they both are the types of vesicles, but the composition of their membranes differs. Niosomes are formed by combining uncharged monomeric surfactants and cholesterol, whereas liposomes are formed by combining neutral or charged double-chain phospholipids. Niosomes are lipid bilayer vesicles composed of nonionic surfactant monomers (82). Nonionic surfactants facilitate the formation of unilamellar and multilamellar vesicles, which serve as cost-effective drug delivery systems exhibiting physical properties similar to liposomes (83). These synthetic monomers spontaneously self-assemble into niosomes upon the addition of water. Niosomes are reported to have higher entrapment efficiency and show excellent metabolic stability (84). The use of niosomes encapsulated with anti-HIV drugs has been demonstrated to effectively overcome conventional drawbacks associated with anti-HIV drugs, such as transport barriers and drug elimination issues (85).
Witika et al. (86) developed an optimized method for the preparation of niosomes for encapsulating NVP, a drug used to treat HIV/AIDS. The niosomes were prepared using a process called thin layer hydration (Figure 7). The production parameters were optimized using design of experiments and response surface methods. The niosomes were prepared with two different types of Span® surfactants (Span® 20 and Span® 80). The parameters such as cholesterol, surfactant content, hydration time, and temperature were optimized. This research aims to characterize niosomes by evaluating key quality characteristics (CQA) such as particle size (PS), entrapment efficiency (EE), polydispersity index (PDI), and the quantity of NVP released after 48 h. The study found that the optimized niosome composition had a mean PS of 523.36 ± 23.16 nm, PDI of 0.386 ± 0.054, EE of 96.8%, and NVP of 25.3% released at 48 h for Span® 20 niosomes, while the mean PS was 502.87 ± 21.77 nm, PDI was 0.394 ± 0.027, EE was 98.0%, and 25.0% NVP was released at 48 h for Span® 80 niosomes. The niosomes were stable for 12 weeks at 4 ± 2 °C and 22 ± 2 °C. Hence, a simple, cheap, rapid, and precise method for manufacturing NVP niosomes and demonstrating the product’s target CQA. Nikravesh et al. (87) enhanced the movement of the anti-HIV drug tenofovir within cells by including the trans-acting activator of transcription (TAT) peptide in a novel PEGylated niosomal formulation (PEG-NI) using the thin-film hydration technique. The researchers conducted a comparison of the morphological and in-vitro effects of tenofovir-loaded TAT-conjugated niosomes (TAT-NI1s) and PEGylated niosomes. The study revealed that the TAT-NI1 with tenofovir had an average size of 208 ± 9.004 nm, a PDI of 0.39 ± 0.008, and an EE of 75 ± 2.516%. The MTT assays yielded the IC50 values, which represent the cytotoxicity levels, for the various formulations as follows: PEG-NI at 65.41 μM, empty PEG-NI at 37.04 μM, TAT-NI1 at 41.02 μM, free Tenofovir at 43.07 μM, and TAT peptide at 37.12 μM. According to the data, it can be concluded that PEG-NI exhibited the highest level of cytotoxicity, while TAT-NI1 demonstrated a moderate level of cytotoxicity compared to the other treatments.
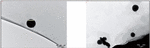
Figure 7. TEM of an optimized Nevirapine (NVP) niosome (86).
Table 3 provides a summary of the advantages and limitations of different types of nanocarriers that can be used for drug delivery. Each nanocarrier has its unique set of advantages and drawbacks, which must be carefully considered when selecting the appropriate carrier for a specific drug delivery application. Dendrimers have an advantage of increased delivery of antiviral siRNA across in vitro BBB but have unpredictable drug release kinetics and can induce cytotoxicity (88). Polymeric micelles have an advantage of increased efficacy for delivering anti-retroviral drugs across in vitro BBB and animal models, but their instability can lead to unpredictable drug release, and they need improvement in selectivity (89). Liposomes naturally penetrate BBB, but they have declined drug EE and require PEGylation to improve stability (90). Niosomes are inexpensive and can entrap drugs efficiently but have a limited shelf life, leading to drug leakage due to hydrolysis, fusion, or leaching, and extensive studies are required (91).
Current challenges and future prospects of nanotechnology in HIV treatment
Nanotechnology holds the potential of novel approaches for HIV treatment; however, it faces several challenges. The recent challenges include the proper delivery of antiretroviral drugs into latent HIV reservoirs, the BBB penetration required to manage HIV-associated neurocognitive disorders effectively, and the biocompatibility and potential toxicity of nanomaterials. A very important challenge remaining is the high cost related to the development of nanotechnology-based therapies, which is a significant barrier to its acceptance and use in resource-constrained settings. Despite these challenges, the prospects for nanotechnology in HIV treatment seem promising, especially with the advancement of targeted drug delivery systems and multifunctional nanocarriers. These innovations can revolutionize HIV therapy by allowing for sustained drug release, reducing side effects, and eliminating latent viral reservoirs, which can advance the pursuit of a functional cure or total eradication of the virus.
Summary
HIV remains a major health concern as there is still no cure available for this deadly disease. Despite significant progress made in the development of antiretroviral therapies, HIV-infected patients must undergo lifelong medication and face the risk of viral resistance. The difficulty in eradicating the virus from the body is due to its ability to integrate into host cells and persist in reservoirs, making complete elimination a significant challenge. Nevertheless, drug delivery methods using nanotechnology have shown remarkable results in overcoming the constraints of traditional HIV therapies. Nanoparticles can effectively target and deliver drugs to specific cells, enhancing drug efficacy while reducing toxicity and side effects. Moreover, they can potentially overcome the blood-brain barrier, which is a significant challenge in treating neuro AIDS, improving drug delivery to the central nervous system. Despite the preclinical stage of nanotechnology-based treatments for HIV, studies have demonstrated their effectiveness in enhancing immune responses, preventing transmission, and improving viral suppression. Further research and development are necessary to overcome the drawbacks of the nanocarriers for clinical practice, leading to the development of effective and efficient HIV therapies.
References
2. Roy, A, and Basak, S. HIV long-term non-progressors share similar features with simian immunodeficiency virus infection of chimpanzees. J Biomol Struct Dyn. (2021) 39:2447–54.
3. Lyall, E, Blott, M, De Ruiter, A, Hawkins, D, Mercy, D, Mitchla, Z, et al. Guidelines for the management of HIV infection in pregnant women and the prevention of mother-to-child transmission: British HIV Association. HIV Med. (2001) 2:314–34.
4. Kapila, A, Chaudhary, S, Sharma, R, Vashist, H, Sisodia, S, and Gupta, A. A review on: HIV aids. Indian J Pharm Biol Res. (2016) 4:69–73.
5. Centers for Disease Control (CDC). Recommendations for assisting in the prevention of perinatal transmission of human T-lymphotropic virus type III lymphadenopathy-associated virus and acquired immunodeficiency syndrome. MMWR Morb Mortal Wkly Rep. (1985) 34:721–6.
6. Crystal, R. Transfer of genes to humans: early lessons and obstacles to success. Science. (1995) 270:404–10.
7. Briggs, J, Wilk, T, Welker, R, Kräusslich, H, and Fuller, S. Structural organization of authentic, mature HIV-1 virions and cores. EMBO J. (2003) 22:1707–15.
8. Dong, X, Liu, L, Zhu, D, Zhang, H, and Leng, X. Transactivator of transcription (TAT) peptide–chitosan functionalized multiwalled carbon nanotubes as a potential drug delivery vehicle for cancer therapy. Int J Nanomed. (2015) 10:3829.
9. Heaphy, S, Finch, J, Gait, M, Karn, J, and Singh, M. Human immunodeficiency virus type 1 regulator of virion expression, rev, forms nucleoprotein filaments after binding to a purine-rich “bubble” located within the rev-responsive region of viral mRNAs. Proc Natl Acad Sci. (1991) 88:7366–70.
10. Kirchhoff, F, Voss, G, Nick, S, Stahl-Hennig, C, Coulibaly, C, Frank, R, et al. Antibody response to the negative regulatory factor (nef) in experimentally infected macaques: correlation with viremia, disease progression, and seroconversion to structural viral proteins. Virology. (1991) 183:267–72.
11. Yang, G, and Xiong, X. Viral infectivity factor: a novel therapeutic strategy to block HIV-1 replication. Mini Rev Med Chem. (2013) 13:1047–55.
12. Deora, A, and Ratner, L. Viral protein U (Vpu)-mediated enhancement of human immunodeficiency virus type 1 particle release depends on the rate of cellular proliferation. J Virol. (2001) 75:6714–8.
Votteler, J, and Schubert, U. Human immunodeficiency viruses: molecular biology. In Encyclopedia of virology. Amsterdam: Elsevier (2008). p. 517–25.
14. Rossi, E, Meuser, M, Cunanan, C, and Cocklin, S. Structure, function, and interactions of the HIV-1 capsid protein. Life. (2021) 11:100.
15. Ran, X, Ao, Z, Trajtman, A, Xu, W, Kobinger, G, Keynan, Y, et al. HIV-1 envelope glycoprotein stimulates viral transcription and increases the infectivity of the progeny virus through the manipulation of cellular machinery. Sci Rep. (2017) 7:1–16.
16. Checkley, M, Luttge, B, and Freed, EO. HIV-1 envelope glycoprotein biosynthesis, trafficking, and incorporation. J Mol Biol. (2011) 410:582–608.
17. Jacobson, K, and Ogbuagu, O. Integrase inhibitor-based regimens result in more rapid virologic suppression rates among treatment-naïve human immunodeficiency virus–infected patients compared to non-nucleoside and protease inhibitor–based regimens in a real-world clinical setting: a retrospective cohort study. Medicine (Baltimore). (2018) 97:e13016.
18. Fitzgerald, N, Cross, M, O’Shea, S, and Fox, J. Diagnosing acute HIV infection at point of care: a retrospective analysis of the sensitivity and specificity of a fourth-generation point-of-care test for detection of HIV core protein p24. Sex Transm Infect. (2017) 93:100–1.
19. Rist, M, and Marino, J. Mechanism of nucleocapsid protein catalyzed structural isomerization of the dimerization initiation site of HIV-1. Biochemistry. (2002) 41:14762–70.
20. Thomas, J, and Gorelick, R. Nucleocapsid protein function in early infection processes. Virus Res. (2008) 134:39–63.
21. Coey, A, Larsen, K, Choi, J, Barrero, D, Puglisi, J, and Puglisi, E. Dynamic interplay of RNA and protein in the human immunodeficiency virus-1 reverse transcription initiation complex. J Mol Biol. (2018) 430:5137–50.
22. Thomas, H, Wilson, S, O’Toole, C, Lister, C, Saeed, A, Watkins, R, et al. Differential maturation of avidity of IgG antibodies to gp41, p24 and p17 following infection with HIV-1. Clin Exp Immunol. (1996) 103:185–91.
23. Balakrishna, L, and Kondapi, A. Role of host proteins in HIV-1 early replication. Adv Mol Retrovirol. (2016) 21.
25. Salimi, H, Johnson, J, Flores, M, Zhang, M, O’Malley, Y, Houtman, J, et al. The lipid membrane of HIV-1 stabilizes the viral envelope glycoproteins and modulates their sensitivity to antibody neutralization. J Biol Chem. (2020) 295:348–62.
26. Santiago, M, Range, F, Keele, B, Li, Y, Bailes, E, Bibollet-Ruche, F, et al. Simian immunodeficiency virus infection in free-ranging sooty mangabeys (Cercocebus atys atys) from the Tai Forest, Cote d’Ivoire: implications for the origin of epidemic human immunodeficiency virus type 2. J Virol. (2005) 79:12515–27.
27. Rife, B, and Salemi, M. On the early dynamics and spread of HIV-1. Trends Microbiol. (2015) 23:3–4.
28. Janoff, E, and Smith, P. Complications of HIV/AIDS and other secondary immunodeficiencies. Yamada’s Textb Gastroenterol. (2022):3105–24.
29. Garg, G, Gogia, A, Kakar, A, and Miglani, P. Persistent marked peripheral eosinophilia due to tuberculosis: a case report. Iran J Med Sci. (2017) 42:102–5.
30. Hernandez-Vargas, E, and Middleton, R. Modeling the three stages in HIV infection. J Theor Biol. (2013) 320:33–40.
31. Benson, C, Brooks, J, Holmes, K, Kaplan, J, Masur, H, and Pau, A. Guidelines for prevention and treatment opportunistic infections in HIV-infected adults and adolescents: recommendations from CDC, the National Institutes of Health, and the HIV medicine association/infectious diseases society of America. MMWR Recomm Rep. (2009) 58:1–207.
32. Wilen, C, Tilton, J, and Doms, RW. HIV: cell binding and entry. Cold Spring Harb Perspect Med. (2012) 2:a006866.
33. Ohlmann, T, Mengardi, C, and López-Lastra, M. Translation initiation of the HIV-1 mRNA. Translation. (2014) 2:e960242.
34. Kwong, P, Wyatt, R, Robinson, J, Sweet, R, Sodroski, J, and Hendrickson, W. Structure of an HIV gp120 envelope glycoprotein in complex with the CD4 receptor and a neutralizing human antibody. Nature. (1998) 393:648–59.
35. Herrera-Carrillo, E, and Berkhout, B. Bone marrow gene therapy for HIV/AIDS. Viruses. (2015) 7:3910–36.
36. Mintz, M. Neurobehavioral manifestations of pediatric AIDS/HIV-1 infection. Pediatr Behav Neurol. (2024) 126:335–65.
Capriotti, Z, and Klase, Z. Innate immune memory in chronic HIV and HIV-associated neurocognitive disorders (HAND): potential mechanisms and clinical implications. J Neurovirol. (2024). doi: 10.1007/s13365-024-01239-2
38. Sanmarti, M, Ibáñez, L, Huertas, S, Badenes, D, Dalmau, D, Slevin, M, et al. HIV-associated neurocognitive disorders. J Mol Psychiatry. (2014) 2:2.
39. Scutari, R, Alteri, C, Perno, C, Svicher, V, and Aquaro, S. The role of HIV infection in neurologic injury. Brain Sci. (2017) 7:38.
Hao, S. Neuropathogenesis of HIV-associated neuropathic pain in HIV-associated neurocognitive disorder. Amsterdam: Elsevier (2024). p. 117–34.
41. Saifullah, M, Laghzaoui, O, Ozyahyalar, H, and Irfan, A. The CRISPR-Cas9 induced CCR5 Δ32 mutation as a potent gene therapy methodology for resistance to HIV-1 variant: a review. Eur Rev Med Pharmacol Sci. (2024) 28:2430–63.
42. Kumar, S, Maurya, V, Dandu, H, Bhatt, M, and Saxena, S. Global perspective of novel therapeutic strategies for the management of neuroAIDS. Biomol Concepts. (2018) 9:33–42.
43. Broder, S. The development of antiretroviral therapy and its impact on the HIV-1/AIDS pandemic. Antiviral Res. (2010) 85:1–18.
44. Jacobson, M, Bacchetti, P, Kolokathis, A, Chaisson, R, Szabo, S, Polsky, B, et al. Surrogate markers for survival in patients with AIDS and AIDS related complex treated with zidovudine. Br Med J. (1991) 302:73–8.
46. Choi, S, Lagakos, S, Schooley, R, and Volberding, P. CD4+ lymphocytes are an incomplete surrogate marker for clinical progression in persons with asymptomatic HIV infection taking zidovudine. Ann Intern Med. (1993) 118:674–80.
47. Randall, P. CPCRA 007: preliminary results of combination antiretroviral study. NIAID AIDS Agenda. (1996) 2.
48. Luma, H, Choukem, S, Temfack, E, Ashuntantang, G, Joko, H, and Koulla-Shiro, S. Adverse drug reactions of Highly active antiretroviral therapy (HAART) in HIV infected patients at the General Hospital, Douala, Cameroon: a cross sectional study. Pan Afr Med J. (2012) 12:87.
49. Hokello, J, Tyagi, K, Owor, R, Sharma, A, Bhushan, A, Daniel, R, et al. New insights into HIV life cycle, Th1/Th2 shift during HIV Infection and preferential virus infection of Th2 cells: implications of early HIV treatment initiation and care. Life. (2024) 14:104.
50. Sailaja, I, Baghel, M, and Shaker, I. Nanotechnology based drug delivery for HIV-AIDS treatment. In AIDS updates-recent advances and new perspectives. London: IntechOpen (2021).
51. Dejucq, N, Simmons, G, and Clapham, P. Expanded tropism of primary human immunodeficiency virus type 1 R5 strains to CD4+ T-cell lines determined by the capacity to exploit low concentrations of CCR5. J Virol. (1999) 73:7842–7.
52. Evering, T, and Markowitz, M. Raltegravir (MK-0518): an integrase inhibitor for the treatment of HIV-1. Drugs Today. (2007) 43:865–78.
53. Branham, M, Ross, E, and Govender, T. Predictive models for maximum recommended therapeutic dose of antiretroviral drugs. Comput Math Methods Med. (2012) 2012:469769.
54. Nowacek, A, and Gendelman, H. NanoART, neuroAIDS and CNS drug delivery. Nanomedicine. (2009) 4:557–74.
55. Lu, C, Zhao, Y, Wong, H, Cai, J, Peng, L, and Tian, X. Current approaches to enhance CNS delivery of drugs across the brain barriers. Int J Nanomed. (2014) 9:2241.
57. Upadhyay, R. Drug delivery systems, CNS protection, and the blood brain barrier. Biomed Res Int. (2014) 2014:869269.
58. Mamo, T, Moseman, E, Kolishetti, N, Salvador-Morales, C, Shi, J, Kuritzkes, D, et al. Emerging nanotechnology approaches for HIV/AIDS treatment and prevention. Nanomedicine. (2010) 5:269–85.
59. Kuo, Y, and Kuo, C. Electromagnetic interference in the permeability of saquinavir across the blood–brain barrier using nanoparticulate carriers. Int J Pharm. (2008) 351:271–81.
60. Jampílek, J, and Kráľová, K. Nanotechnology: new frontiers in anti-HIV therapy. In Rai M and Yadav A editors. Nanotechnological application virology. Amsterdam: Elsevier Science (2022). p. 129–71.
61. Mahajan, S, Roy, I, Xu, G, Yong, K-T, Ding, H, Aalinkeel, R, et al. Enhancing the delivery of anti retroviral drug “Saquinavir” across the blood brain barrier using nanoparticles. Curr HIV Res. (2010) 8:396–404.
62. Patra, J, Das, G, Fraceto, L, Campos, E, Rodriguez-Torres, MP, Acosta-Torres, LS, et al. Nano based drug delivery systems: recent developments and future prospects. J. Nanotechnol. (2018) 16:1–33.
63. Chonn, A, and Cullis, P. Recent advances in liposomal drug-delivery systems. Curr Opin Biotechnol. (1995) 6:698–708.
65. Torchilin, V. Recent advances with liposomes as pharmaceutical carriers. Nat Rev Drug Discov. (2005) 4:145–60.
66. Oussoren, C, Magnani, M, Fraternale, A, Casabianca, A, Chiarantini, L, Ingebrigsten, R, et al. Liposomes as carriers of the antiretroviral agent dideoxycytidine-5′-triphosphate. Int J Pharm. (1999) 180: 261–70.
67. Bronshtein, T, Toledano, N, Danino, D, Pollack, S, and MacHluf, M. Cell derived liposomes expressing CCR5 as a new targeted drug-delivery system for HIV infected cells. J Control Release. (2011) 151:139–48.
68. Kenchappa, V, Cao, R, Venketaraman, V, and Betageri, GV. Liposomes as carriers for the delivery of efavirenz in combination with glutathione—an approach to combat opportunistic infections. Appl Sci. (2022) 12:1468.
69. Kheoane, P, Enslin, G, and Tarirai, C. Formulation and characterization of liposomes containing drug absorption enhancers for optimized anti-HIV and antimalarial drug delivery. Drug Deliv Transl Res. (2023) 13:1358–71.
70. Nagasaki, Y, Yasugi, K, Yamamoto, Y, Harada, A, and Kataoka, K. Sugar-installed block copolymer micelles: their preparation and specific interaction with lectin molecules. Biomacromolecules. (2001) 2:1067–70.
71. Ray, S, Seth, S, Bhattacharyya, U, and Sen, M. Polymeric micelles for theranostic uses. Des Appl Theranostic Nanomed. (2023):109–24.
72. Orenstein, J, Fox, C, and Wahl, S. Macrophages as a source of HIV during opportunistic infections. Science. (1997) 276:1857–61.
73. Saravanan, M, Asmalash, T, Gebrekidan, A, Gebreegziabiher, D, Araya, T, Hilekiros, H, et al. Nano-medicine as a newly emerging approach to combat Human Immunodeficiency Virus (HIV). Pharm Nanotechnol. (2018) 6:17–27.
74. Chiappetta, D, Hocht, C, Opezzo, J, and Sosnik, A. Intranasal administration of antiretroviral-loaded micelles for anatomical targeting to the brain in HIV. Nanomedicine (Lond). (2013) 8:223–37. doi: 10.2217/nnm.12.104
75. Chiappetta, D, Facorro, G, Rubin de Celis, E, and Sosnik, A. Synergistic encapsulation of the anti-HIV agent efavirenz within mixed poloxamine/poloxamer polymeric micelles. Nanomed Nanotechnol Biol Med. (2011) 7:624–37.
76. Lamrayah, M, Phelip, C, Coiffier, C, Lacroix, C, Willemin, T, Trimaille, T, et al. A Polylactide-based micellar adjuvant improves the intensity and quality of immune response. Pharmaceutics. (2022) 14:107.
77. Kedar, P, Saraf, A, Maheshwari, R, and Sharma, M. Advances in dendritic systems and dendronized nanoparticles: paradigm shifts in cancer targeted therapy and diagnostics. Mol Pharm. (2024) 22:28–57.
78. Sepulveda-Crespo, D, Gomez, R, De La Mata, F, Jimenez, J, and Muñoz-Fernández, MÁ. Polyanionic carbosilane dendrimer-conjugated antiviral drugs as efficient microbicides: recent trends and developments in HIV treatment/therapy. Nanomed Nanotechnol Biol Med. (2015) 11: 1481–98.
79. Sarode, R, and Mahajan, H. Dendrimers for drug delivery: an overview of its classes, synthesis, and applications. J Drug Deliv Sci Technol. (2024) 98:105896.
80. Kumar, P, Kumar, P, Anneer Selvam, T, and Rao, K. Prolonged drug delivery system of PEGylated PAMAM dendrimers with a anti-HIV drug. Res Pharm. (2015) 3.
81. Royo-Rubio, E, Rodríguez-Izquierdo, I, Moreno-Domene, M, Lozano-Cruz, T, de la Mata, FJ, Gómez, R, et al. Promising PEGylated cationic dendrimers for delivery of miRNAs as a possible therapy against HIV-1 infection. J Nanobiotechnol. (2021) 19:1–16.
82. Patel, P, Parashar, A, Kaurav, M, Yadav, K, Singh, D, Gupta, G, et al. Niosome: a vesicular drug delivery tool. In Nanoparticles and nanocarriers based pharmaceutical preparations. Sharjah: Bentham Science Publishers (2022). p. 333–64.
83. Palash, A, Esha, R, Kiran, P, and Javid, S. Development of HIV therapeutic approaches based on nanotechnology. Res J Biotechnol. (2024) 19.
84. Alkilani, A, Sharaire, Z, Hamed, R, and Basheer, H. Transdermal delivery system of doxycycline-loaded niosomal gels: toward enhancing doxycycline stability. ACS Omega. (2024) 9:33542–56.
85. Malik, T, Chauhan, G, Rath, G, Kesarkar, R, Chowdhary, A, and Goyal, A. Efaverinz and nano-gold-loaded mannosylated niosomes: a host cell-targeted topical HIV-1 prophylaxis via thermogel system. Artif Cells Nanomed Biotechnol. (2018) 46:79–90.
86. Witika, B, and Walker, R. Development, manufacture and characterization of niosomes for the delivery for nevirapine. Die Pharm Int J Pharm Sci. (2019) 74:91–6.
87. Yadavar-Nikravesh, M, Ahmadi, S, Milani, A, Akbarzadeh, I, Khoobi, M, Vahabpour, R, et al. Construction and characterization of a novel tenofovir-loaded pegylated niosome conjugated with tat peptide for evaluation of its cytotoxicity and anti-HIV effects. Adv Powder Technol. (2021) 32:3161–73.
88. Rastogi, V, Yadav, P, Porwal, M, Sur, S, and Verma, A. Dendrimer as nanocarrier for drug delivery and drug targeting therapeutics: a fundamental to advanced systematic review. Int J Polym Mater Polym Biomater. (2024) 73: 310–32.
89. Kharwade, R, and Mahajan, N. Role of nanocarriers for the effective delivery of anti-HIV drugs. In Mahajan NM, Saini A, and Rahut N , editors. Photophysics and nanophysics in therapeutics. Amsterdam: Elsevier (2022). p. 291–310.
90. Patel, R, Patel, A, Trivedi, N, Pandya, V, Alexander, A, Patel, V, et al. Liposomes as carrier for drug delivery in Alzheimer’s disease. In Prajapati BG, Chellappan D, and Kendre P editors. Alzheimer’s disease and advanced drug delivery strategies. Cambridge, MA: Academic Press (2024). p. 153–79.
91. Kandpal, R, Joshi, A, Kumar, K, Rajput, V, and Chauhan, V. An updated review on niosomes: a promising drug carrier. Int J Indig Herbs Drugs. (2023) 8:53–7.
92. Chiappetta, D, Hocht, C, Taira, C, and Sosnik, A. Oral pharmacokinetics of the anti-HIV efavirenz encapsulated within polymeric micelles. Biomaterials. (2011) 32:2379–87.
© The Author(s). 2024 Open Access This article is distributed under the terms of the Creative Commons Attribution 4.0 International License (https://creativecommons.org/licenses/by/4.0/), which permits unrestricted use, distribution, and reproduction in any medium, provided you give appropriate credit to the original author(s) and the source, provide a link to the Creative Commons license, and indicate if changes were made.